4 Self- / Non-Self-Recognition and Local Adaptation
evolution, coevolution, host-parasite interactions, Muller’s ratchet, parthenogenesis, Red Queen hypothesis, sexual reproduction
One way to falsify the Red Queen is to experimentally show that the expectations of the hypothesis are not met. One expectation is that parasites would quickly become adapted to infecting their local host populations. Here is the logic. If parasites are closely tracking common host genotypes in their local (sympatric) populations, then they should be better, on average, at infecting sympatric hosts than foreign (allopatric) hosts. If this is not the case, then parasites would seem unlikely (at least to me) to be a factor selecting for sexual reproduction.
I am often asked why we would expect the parasites to be better at infecting their local hosts instead of the opposite. Why shouldn’t hosts evolve to be more resistant to their local parasites than to allopatric parasites? It is a fair question. One common answer is that parasites are locally adapted to host populations because they have faster generation times. But that cannot be the whole answer. Theory has shown that parasites can be locally adapted even when there is no generation-time asymmetry (Gandon & Michalakis 2002; Lively 1999). Instead, I think the answer has more to do with the underlying genetic basis for infection.
What, then, is the genetic basis for infection? This was unknown, but I was assuming that all animal hosts have a self- / non-self-recognition system, such that they can detect foreign tissues (e.g., parasites or tissue grafts) that do not match their own. Sponges, for example, accept tissue grafts from self, but reject grafts from unrelated individuals of the same species (review in Gaino et al. 1999). This ability to reject foreign tissues seems widely conserved (Buss 1990). I was also assuming that the self- / non-self-recognition system is genetically variable and that different host genotypes would dominate in different populations. Parasite genotypes that match the most common local host genotypes would be favored by natural selection, and these parasite genotypes should increase in frequency. This should lead to local adaptation by the parasites. Fortunately, one can test for local adaptation using reciprocal cross-inoculation experiments.
4.1 Experimental Studies of Local Adaption
While I was still a post-doc in New Zealand, I set up two reciprocal cross-inoculation experiments to test for local adaptation by the parasites. I knew from my field surveys that one species of sterilizing trematode was especially common in lake populations of the snail. This species was not formally described, but Jan McKenzie sent it to a trematode expert in France, who thought it belonged in the genus Microphallus; hence I will refer to it as Microphallus sp.1 The life cycle of Microphallus turns out to be especially crucial to the story. The adult worms are tiny simultaneous hermaphrodites that live in the intestines of ducks. They cross-fertilize and produce eggs that are shed with the duck feces into the environment. In most trematodes, the eggs normally hatch in water, thereby releasing a swimming larval stage (miracidia), which actively swims to and penetrates the body of snails. This is the case for the trematodes that cause the human disease, Schistosomiasis. But, in this New Zealand species of Microphallus, the eggs hatch not in the environment but rather after being ingested by snails. The larvae then penetrate the snail from the inside. If the snail’s immune system does not recognize the larvae as foreign tissue, the larvae reproduce asexually, producing several hundred cysts (metacercaria) in the snail. These cysts completely replace the reproductive tissue in both males and females. Infected snails are sterilized (Figure 4.1; Figure 4.2).
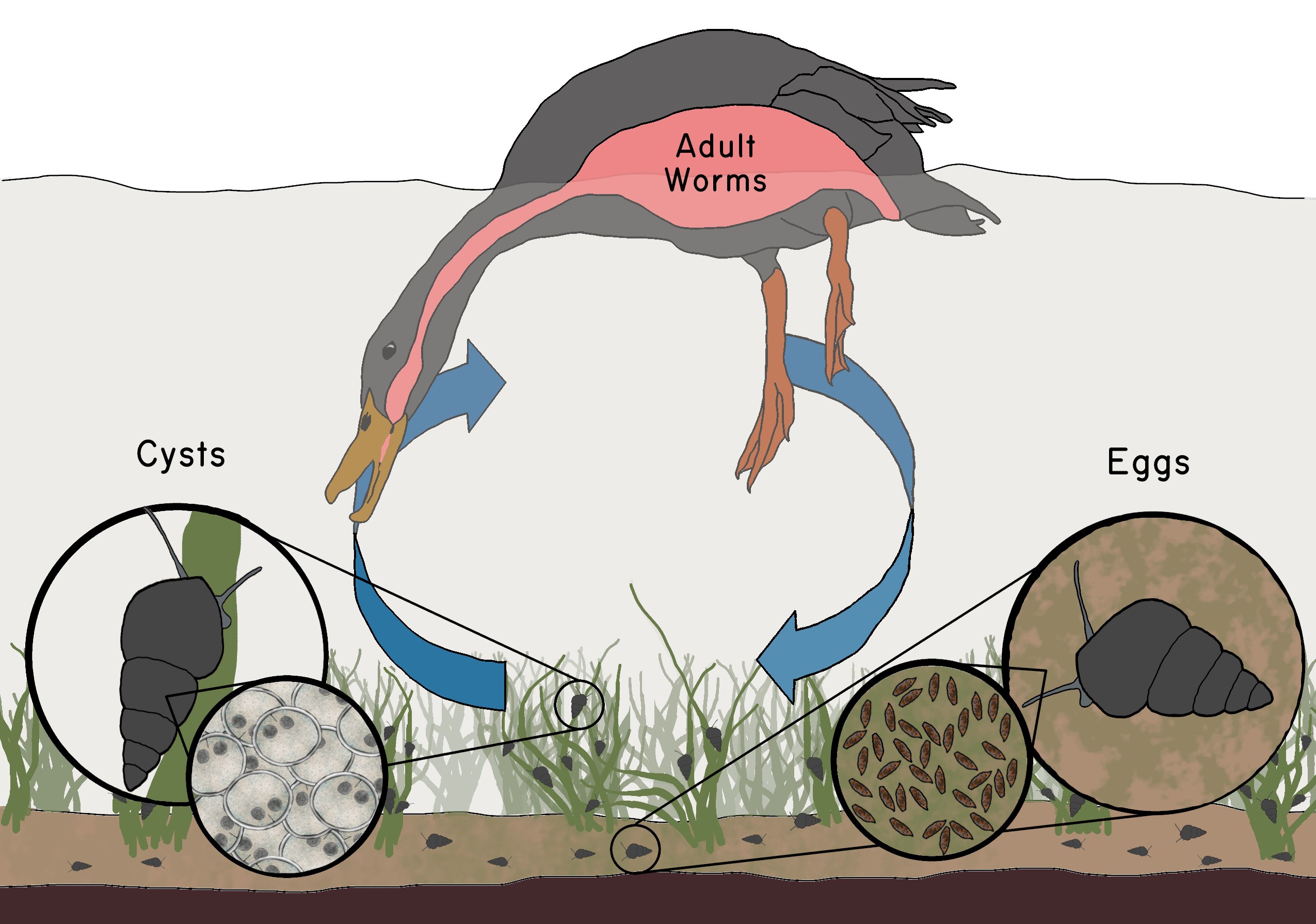
hatchand mature following ingestion by ducks, thus completing the life cycle. Drawing by Zoe M Dinges.
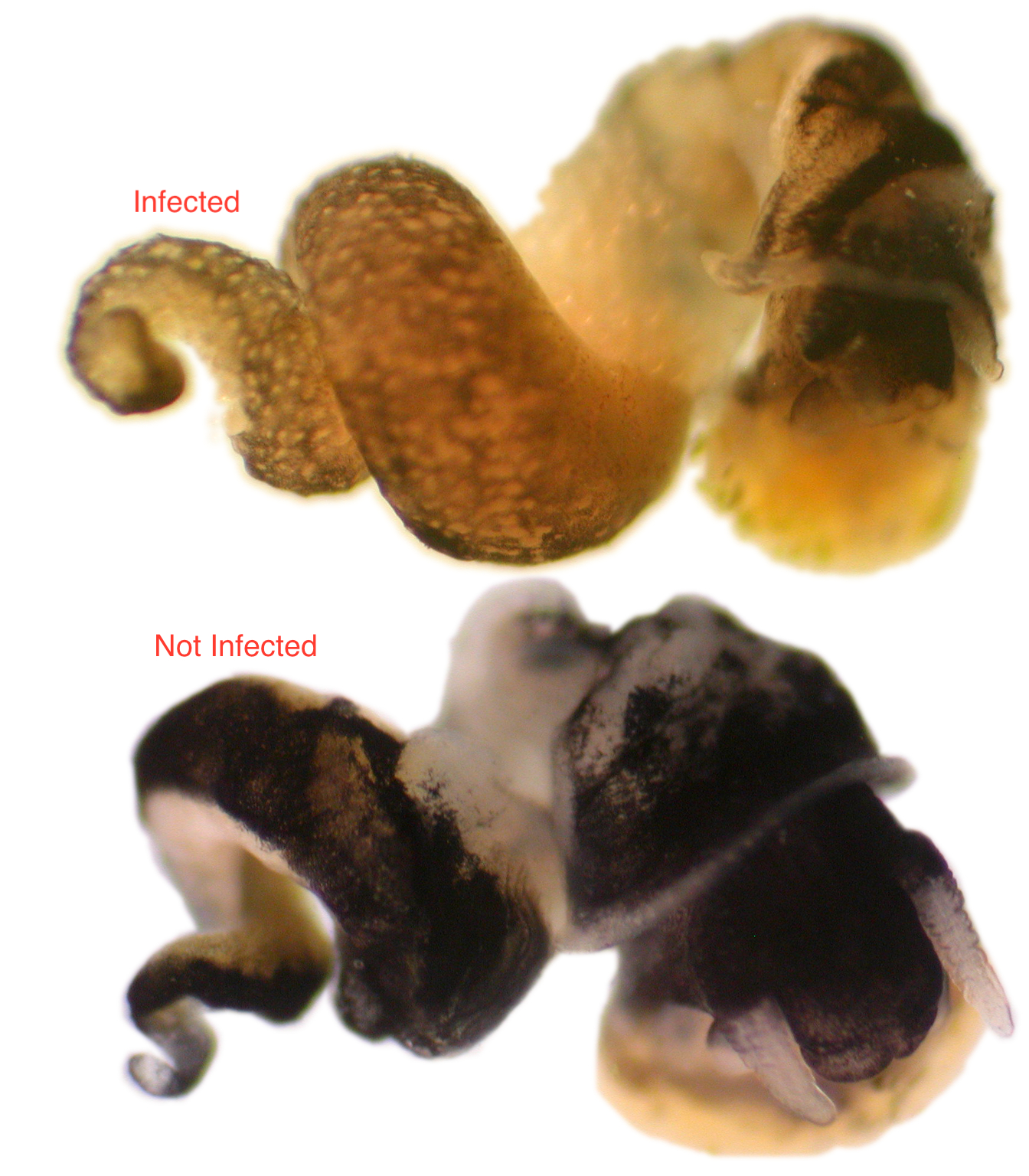
I set up an experiment in which I exposed snails from two different lakes to Microphallus eggs from both lakes. One lake was on the east side of the Southern Alps (Lake Alexandrina), and the other lake was on the west side of the alps (Lake Mapourika). I reasoned that gene flow between the two lakes was likely to be very low, so it seemed unlikely that gene flow could swamp out parasite-mediated selection (if present). But to get the eggs, I had to first complete the life cycle of the parasite in the lab. This had never been done. Eventually, I took the advice of Dr. David Blair, a friend and parasitologist at the University of Canterbury: I fed the cysts to lab mice. I then collected the mouse poo, washed it, and fed the slurry to snails. It seemed very unlikely to work, as ducks (not mice) were the vertebrate host, but I tried it anyway. It worked! I was able to experimentally infect snails in the lab. And, amazingly, the parasites from both lake populations were much more infective to snails from their same lake (Figure 4.3). In other words, the Microphallus parasites were locally adapted (Lively 1989). There was no reason based on this experiment to discard the Red Queen.
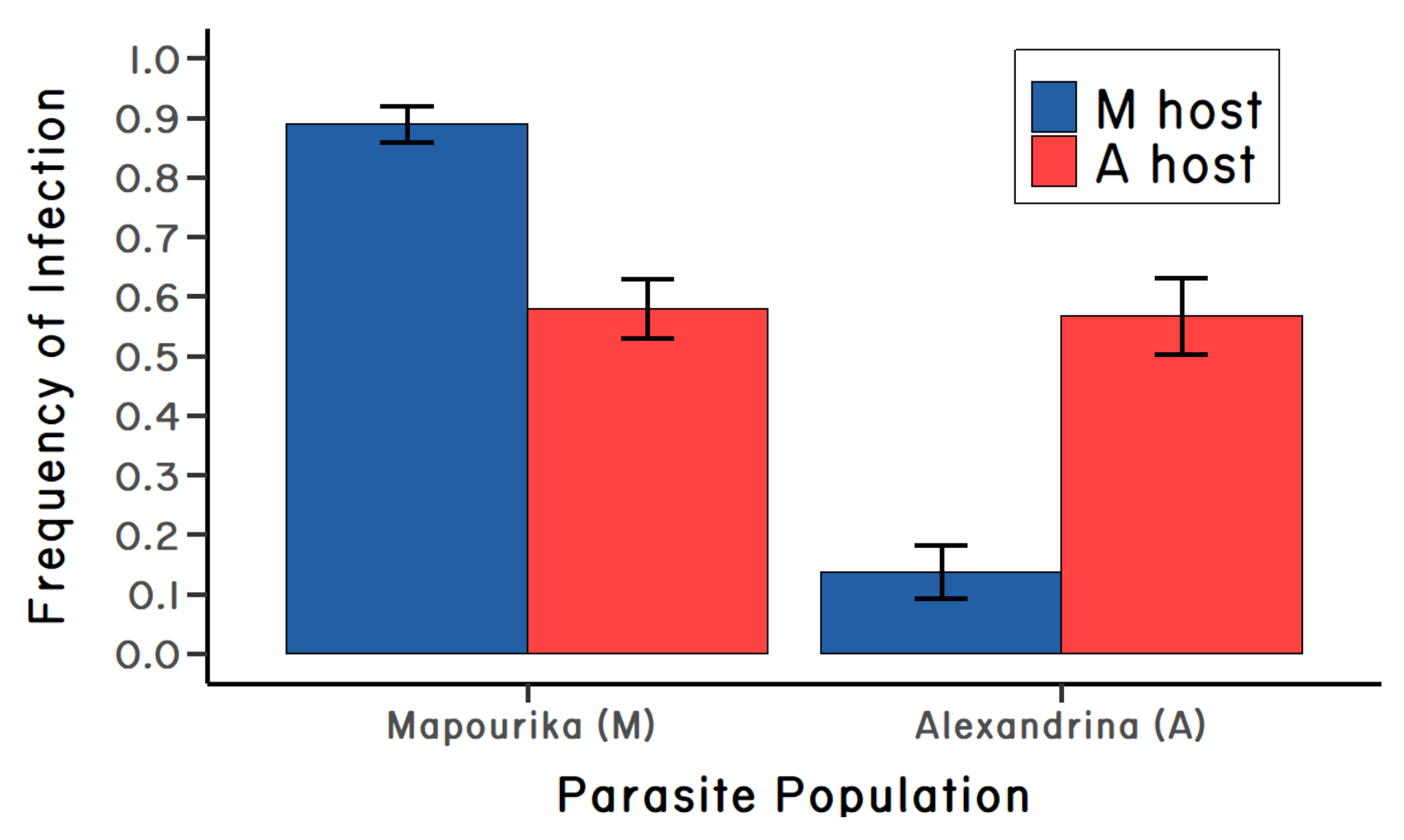
On the other hand, there were only two lakes in the experiment, so it was unclear if local adaptation was generally true for Microphallus. So, I repeated the experiment (Lively 1989). This time I sampled three lakes, all on the west side of the alps. Two of the lakes were less than ten kilometers apart (Lakes Mapourika and Wahapo). The third lake (L. Paringa) was about 100 kilometers south of Lake Mapourika (see map in Lively (1989)). It seemed likely to me that parasite gene flow would be very high between Mapourika and Wahapo, which might reduce or eliminate local adaptation in the parasite. I exposed snails from all three lakes to parasites from each of the three lakes in a fully reciprocal cross-inoculation experiment. Again, the results were very clear: the parasites (following passage through mice) from all three lakes were more infective to host snails from the same lake (Lively 1989). And, to my surprise, the distance between lakes did not matter to the strength of local adaptation (Figure 4.4). Taken together the results from two independent experiments showed strong adaption by parasites to local populations of their snail host. The results also suggested a genetic basis for host resistance and parasite infectivity, which is a crucial assumption of the Red Queen Hypothesis. Finally, the pattern of local parasite adaptation in the snail-trematode system would be found to be very robust in experiments conducted by my students after I moved to Indiana University (Figure 4.5).
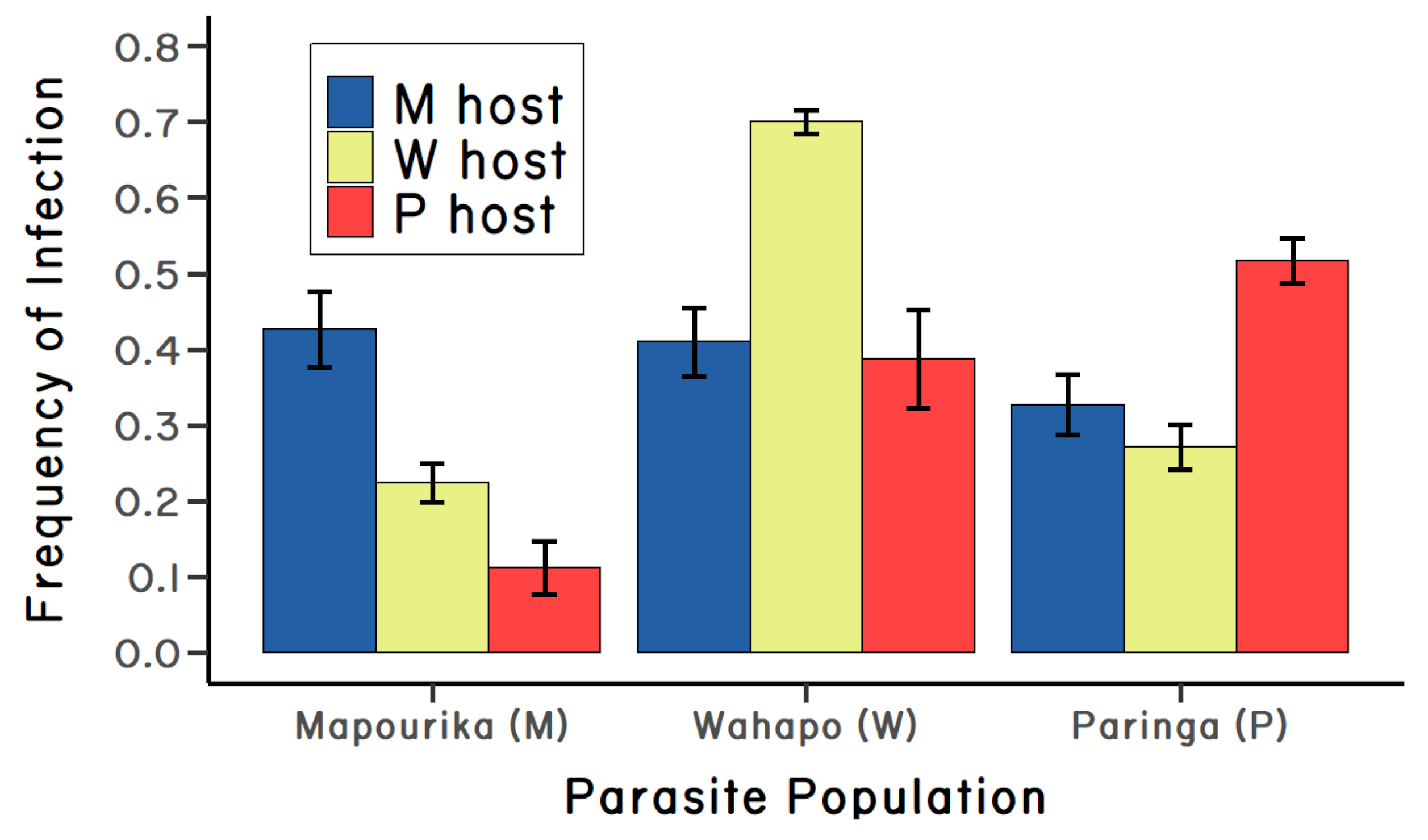
Does local adaptation by parasites mean that hosts are losing? If so, are hosts maladapted, as often claimed? No and no. The host population is evolving as fast as it can to resist infection by local parasites, and local parasites are tracking the genetic changes in their host populations (with a time lag). This tracking by parasites results in local adaption. In computer simulations, the change in host mean fitness oscillates over time from positive to negative and back. Parasite mean fitness also oscillates from positive to negative, but it is 180 degrees out of phase with the host (Lively 1999; Lively & Wade 2022). Thus, local parasite adaptation does not necessarily mean that the hosts are losing. Sometimes the host is winning (positive change in mean fitness) and the parasite is losing (negative change in mean fitness), and sometimes the host is losing and the parasite is winning. Over time, the average change in mean fitness for both species is zero.
My early results on local adaptation along with the results of Parker (1985) and Ebert (1994) convinced me that host-parasite coevolution was interesting, whether or not it could explain sex.2 In addition, May and Anderson (1983) had already ignited a general interest in parasites. They showed that, contrary to conventional wisdom, parasites would evolve to maximize their own rates of transmission without any consideration
for the well-being of their hosts. This work, combined with Hamilton and Zuk’s model of parasite-mediated mate choice, along with Hamilton’s models on parasite-mediated selection for sex, lit up the field (Hamilton 1980; Hamilton & Zuk 1982). Fascinating work by Janice Moore (1984) on the evolution of parasite-mediated modifications of host behavior piled on. In addition, Hudson et al. (1998) rocked the ecological world with an experiment showing that oscillations in Red Grouse densities were controlled by nematode infections. Parasites were now on the map. An emerging new field called ecology and evolution of infectious disease
was taking flight.
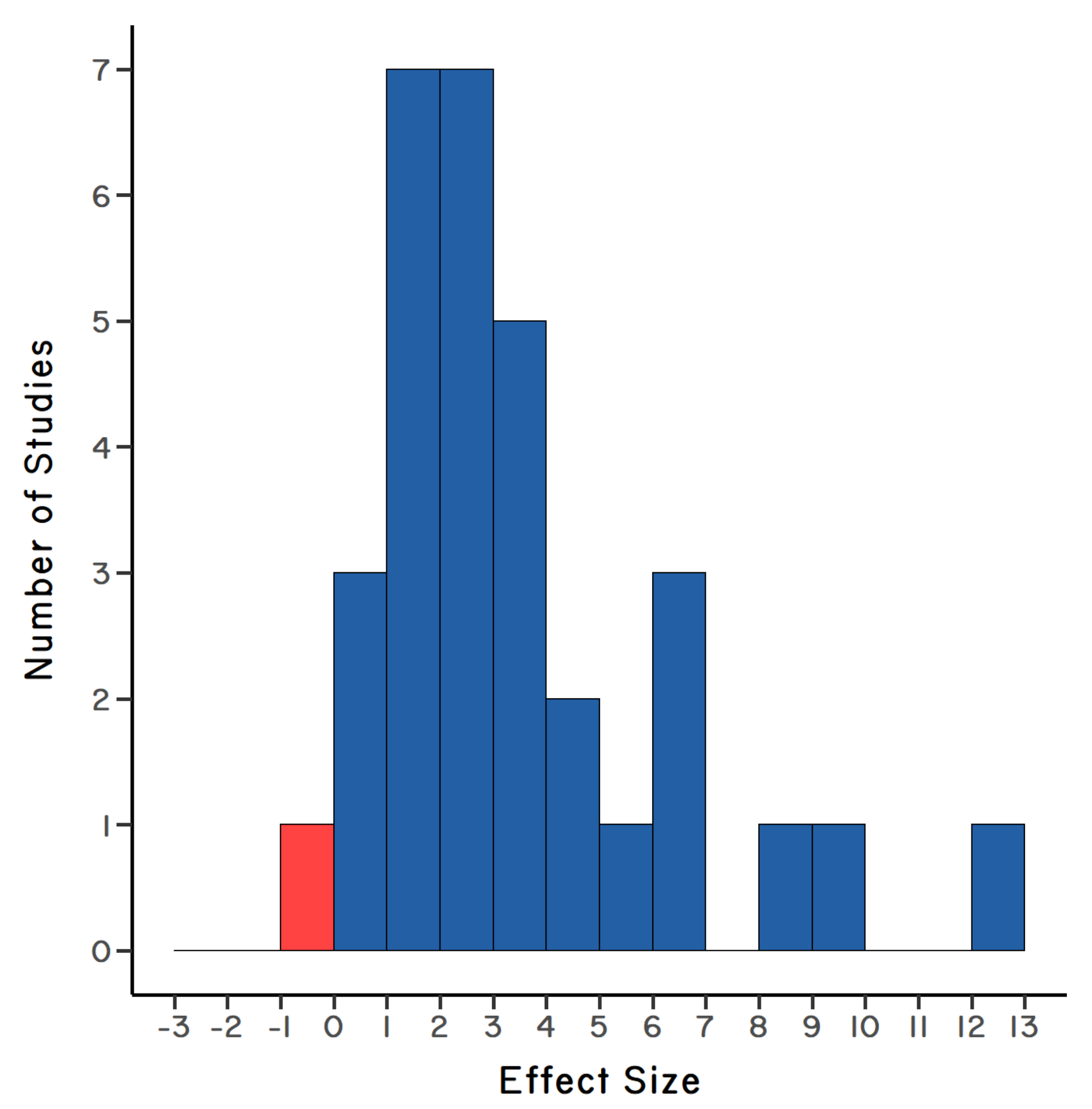
effect sizedepends on the magnitude of the difference between the proportion of infection in sympatric versus allopatric hosts, where positive values indicate that the parasite is adapted to infecting snails from the local host population. Large effect sizes are indicative of stronger local adaptation by parasites. Positive effect sizes are shown in blue; negative effect sizes are shown in red. A statistical test on the effect sizes showed that, in general, the parasite is strongly adapted to infecting local populations of its host. There were 32 total sympatric/allopatric comparisons. (Redrawn from Lively et al. 2004).
4.2 Self- / Non-Self-Recognition
In my presentation thus far, I have been assuming that the molecules on the cell surfaces of parasites must mimic host molecules to evade detection. Otherwise, the parasites will be identified as foreign and killed by the host. In other words, there is a self- / non-self-recognition system in hosts, which is determined by a polymorphic set of alleles at one or more loci.
Where did this idea for self- / non-self-recognition come from? I am embarrassed to say that I did not know before writing this chapter, and I am still not exactly sure, but the idea goes back at least to Sir Frank McFarland Burnet, who was cowinner of the 1960 Nobel Prize in Physiology and Medicine (with Sir Peter Medawar). Burnet was reviewing two studies, which showed recognition and rejection of genetically different competitors of the same species (Burnet 1971). The first study was from Hidemiti Oka (1970): Here I shall explore the possibility that Oka’s (1970) studies of colonial tunicates (Botryllus) … may throw light on primitive types of
Hidemiti Oka was a Japanese professor of biology in Tokyo. He was following up on a pioneering study by Bancroft (1903), who had shown that fragments from related colonies of a compound ascidian (Botryllus schlosseri) readily fused, whereas fragments from unrelated colonies rejected each other. Oka’s goal was to understand the genetic basis for this fusion/rejection. His study suggested that fusion was determined by multiple alleles (self and not-self
recognition from which adaptive immunity may have evolved.perhaps several scores
) at a single locus. More specifically, Oka’s results suggested that fusion occurred between different colonies only if they shared at least one allele at this locus.3 To my mind, this was a major finding.
It thus appears that Oka was inspired by Bancroft, and Burnet was inspired by Oka. How does Burnet fit in? Burnet first accepts Oka’s assumption that allorecognition depends on a single highly polymorphic locus (Figure 4.6): To summarize Oka’s work, it is convenient to accept his assumption, which is validated by much preliminary work, that fusion or rejection between colonies depends on a single locus with many alleles which can be referred to as recognition genes.
Burnet then suggests that this same mechanism could be co-opted as a defense against parasites and that such a mechanism could be the progenitor of the more sophisticated adaptive immune system in vertebrates. Burnet (1971) writes:
It is probably unwise to attempt to imagine the various steps by which such changes could be made. One can foresee a period of great research activity in these fields of tissue fusion and rejection in invertebrates and protochordates during the next decade. Undoubtedly a variety of intriguing phenomena will be uncovered, differing from group to group. Some may be further along the road toward adaptive immunity than the colonial ascidians. Much more extensive comparative studies are called for and in due course analysis of the results should allow a clear evolutionary history to emerge. Whatever form that history eventually takes we can be certain that gene duplication (gene expansion) plays a major part, and that progressive specialization of cell function and phenotypic restriction will be as conspicuous as it is in all other organs and functions. Copyright © 1971, Springer Nature Limited
Wow. In retrospect, Burnet’s attempt to imagine the various steps
does not seem at all unwise. Importantly, he suggests that infection occurs when parasites have alleles that match their host’s genotype. Otherwise, the parasites are killed. This is the logic of what will later be called the matching alleles model
for infection.
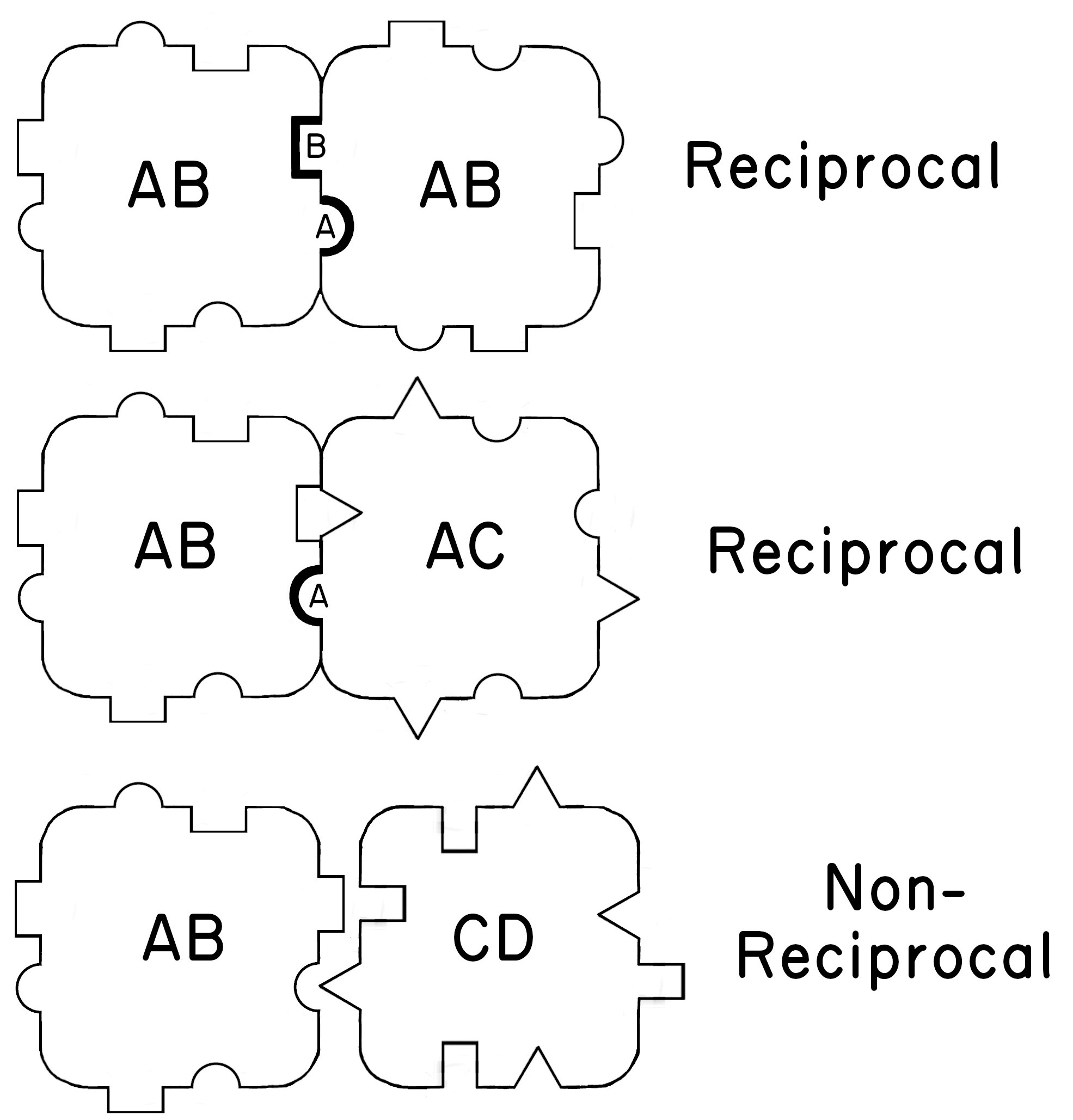
reciprocalfor matching cells with at least one matching allele, and
non-reciprocalfor cells in which none of the alleles matched. Redrawn from Burnet (1971) by Zoe M Dinges.
4.3 Summary
- The most common trematode infection in lake populations of P. antipodarum is caused by a larval stage of Microphallus sp. (recently renamed to Atriophallophorus winterbourni). The trematode has a two-host life cycle (snails and waterfowl).
- Experimental cross-inoculation experiments showed that Microphallus is more infectious to local lake populations of its snail host, P. antipodarum, than to remote host populations.
- These results suggest that some kind of match by the parasite to the host is required for infection. This idea of matching is consistent with the idea of self- / non-self-recognition as the basis for immune defense. The gist of the original idea stems from experimental studies of colony fusion in tunicates.
The trematode worm was not formally described until 30 years later (Blasco-Costa et al. 2019). As it turns out, it belongs in the genus Atriophallophorus, rather than Microphallus, and it was very appropriately named after Mike Winterbourn: A. winterbourni. But I am going to call it Microphallus in this book, as that is what we called it in our early papers.↩︎
Dieter Ebert showed that parasites of Daphnia were locally adapted for both infectivity and transmission, which was a major advance.↩︎
In direct contrast, however, Oka found that cross-fertilization did not occur between Botryllus gametes that shared the same allele. As Oka noted, this result mirrors the S-allele system in plants:
The … situation corresponds exactly in its form to the homomorphic self-incompatibility prevailing among angiosperms.
↩︎